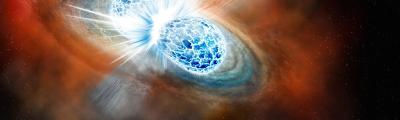
Two neutron stars collided in space, unleashing a deadly beam of gamma rays, spewing heavy metals into the cosmos and drawing the attention of over 70 observatories worldwide. The universe doesn’t get more breathtaking.

An artist’s concept of what a neutron star collision would look like. Credit: Illustration by Robin Dienel courtesy of the Carnegie Institution for Science>
Sandhya Ramesh is a science writer focusing on astronomy and earth science.>
Astrophysicists today have released no less than seven papers on one of the biggest events not only reported in the media but also in the known universe. For the first time ever, we have definitive evidence of two neutron stars colliding and releasing a deadly gamma-ray burst. The light from this burst arrived almost simultaneously with gravitational waves unleashed by the collision.>
This discovery is proof that gravitational waves travel at the speed of light – a prediction that Albert Einstein made over a 100 years ago. Moreover, follow-up observations in the days following the first offered proof that over half the elements heavier than iron are created in such cataclysmic events in the universe.>
Earlier this year, the physicist trio of Rainer Weiss, Kip Thorne, and Barry Barish won the Nobel Prize for physics for their contributions to building the gravitational wave detector called LIGO and its first direct detection of gravitational waves in 2015.>
According to Einstein’s theory of general relativity, when two massive bodies accelerate or collide with each other, they release gravitational energy. Such energy deforms the spacetime fabric as it travels outwards from the source at the speed of light. Imagine ripples radiating outwards when a rock hits the surface of a still pond. LIGO (Laser Interferometer Gravitational-wave Observatory) is made of two detectors located in the US. It observed one set of waves in September of 2015, when two black holes orbited each other faster and faster, coming closer until they collided. The event occurred 1.4 billion lightyears away and let loose 178.7 billion trillion trillion trillion joules of gravitational energy>
LIGO then detected another black hole merger from approximately the same distance away in December 2015. A third observation followed in January 2016, of two black holes that collided 2.9 billion lightyears away. The fourth was made in August 2017, of two black holes colliding 1.8 billion lightyears away. LIGO’s ‘listening’ in on these collisions has helped scientists establish that black holes merging to form bigger black holes is quite a common occurrence in the universe.>
LIGO’s success spurred the creation of more observatories, setting the stage for a new kind of astronomy altogether. In 2016, the Virgo detector in Italy – a collaborative effort by Italy, France, the Netherlands, Poland, Hungary and Spain – was revamped to become more sensitive than before. It joined the two LIGO detectors to hunt for gravitational waves. All three of them are ‘advanced’ detectors and observed the fourth wave on August 14 this year.>
But then, only three days later, the trifecta detected a fifth set of waves, the strongest yet. And exactly two seconds later, two telescopes orbiting Earth saw flash of a gamma-rays from the same point in space. It wasn’t from black holes.
What was different this time?>
The first four observed gravitational waves were all caused by two black holes rapidly orbiting each other and eventually colliding, forming a new and larger black hole. However, black hole mergers are not the only events that can produce gravitational waves.
Black holes are part of a group called compact objects: they are the remains of a star after it has died. The two other main compact objects are white dwarfs and neutron stars. The former is formed when a star up to eight times the mass of our Sun expands as it grows old and sheds its outer layers, retaining only the core. A neutron star is formed when a star that weighs more than eight- to thirty-times the solar mass explodes in a supernova. (By the way, supernovae also produce gravitational waves).>
Neutron stars are the smallest and densest stars in the universe (even though they don’t make their own energy). Such an object will weigh 1.5-2 times the Sun but will measure only 10-20 km wide. A teaspoon of neutron star material would be heavier than Mt Everest; a can of Coke will weigh more than all the humans on Earth combined. The particles in this structure are so tightly packed together that protons and electrons merge to form neutrons. So these objects are almost entirely made up of neutrons. They also spin rapidly, creating extremely powerful magnetic fields around themselves.
Also read: Gravitational waves will bring the extreme universe into view>
On August 17, just like the previous binary black holes, LIGO witnessed two neutron stars spinning around each other, accelerating, before finally making contact – although it was anything but gentle. This process unleashed gravitational waves, some of which LIGO detected. This official name of this collision is GW170817.>
Two seconds after the waves were produced, the resulting merger released a gamma-ray burst (GRB). GRBs are one of the most powerful explosions in the universe. In 10 seconds, they liberate as much energy as our Sun would in 10 billion years. The burst is being called GRB170817A.>
GRBs are also the brightest electromagnetic events known to occur. They can be seen with the naked eye even if they are billions of lightyears away. This is because the energy in a GRB is released in the form of a beam of radiation: it is focused and directional, like laser. Thus, it preserves enough energy to travel across billions of lightyears, even as its source’s radiation (other forms) can’t be seen by us at all. If a GRB were to light up in the Milky Way and aim straight for us, our planet will be fried, wiping out all humanity. But don’t worry yet our galaxy doesn’t host any stars massive enough to pose this threat.>
While scientists had known that GRBs are emitted when massive stars collapse to form a black hole, they’d only theorised that GRBs would also appear when neutron stars collide. The two space telescopes that confirmed the first observation were NASA’s Fermi Gamma-ray Space Telescope and ESA’s INTErnational Gamma Ray Astrophysics Laboratory (INTEGRAL).>
The tricky part about observing GRBs is zeroing in on the exact location of the source. We can detect a flash of light but the only way to find out what caused it is to observe the afterglow: signals that reach us later, like X-ray, infrared, ultraviolet, and microwave radiation. And in the initial days of GRB observations, the bursts disappeared before telescopes could turn to look at the source. But today, we have a slew of telescopes actively searching for GRBs that are also ready to jump in to observe the afterglow from one. The afterglow of GW170817/GRB170817A was observed for 10 days in X-ray, optical, radio-frequency and infrared light. And they also had help from the ground.>
As soon as the gravitational waves hit us, several large telescopes in Chile were asked to look at the patch of sky, towards the Hydra constellation. After a few hours, the Swope Telescope first saw a prick of light in the NGC 4993 galaxy just as the European Southern Observatory’s Visible and Infrared Survey Telescope for Astronomy (VISTA) saw infrared radiation from the same point. As night fell across different countries, more telescopes pointed towards NGC 4993, including India’s AstroSat, Giant Metrewave Radio Telescope (Pune), and the Himalayan Chandra Telescope (Hanle). Many of them picked up the source in their own wavelengths, confirming that the gravitational waves and the GRB did indeed originate from a binary neutron star merger in the galaxy.>
Over the next few weeks, NGC 4993 became the target of several independent observations. Nearly 70 observatories around the world observed the afterglow, resulting in one of the biggest scientific collaborations in modern astronomy. And at its end, scientists concluded that the merger had produced both gravitational waves and electromagnetic radiation, followed by a gamma-ray burst, 130 million lightyears away. So this the closest source of gravitational waves and GRBs we’ve spotted thus far.>

An infographic showing the sequence of events associated with GW170817/GRB170817A. Credit: C. Evans/K. Jani/Georgia Tech>
The explosion that follows a neutron star merger is called a kilonova, and astronomers have finally observed one after it was predicted nearly three decades ago. A kilonova is a radioactive expulsion of heavy metals like selenium, ruthenium, gold and platinum, flung out into space at nearly 20% the speed of light (about 60,000 km/s). The GW170817/GRB170817A kilonova, which ejected almost a 100 billion billion billion kg of material, was observed to contain the elements caesium and tellurium.>
Also read: Search for actual source of plutonium isotope also sets cap on gravitational wave detection>
Astrophysicists have believed for a while that over half the elements heavier than iron in the periodic table are born in neutron-star mergers. These heavy elements are created through a process called rapid neutron capture: free neutrons attach themselves to any heavy nuclei and form even heavier elements. The nuclei of these heavier elements then spread out and infiltrate all nearby galaxies, getting into the dust and clouds that form other stars and planets. “Every bit of gold we mine on our planet and put in the ornaments we wear has traveled to us from the collision of such neutron stars millions of light years away,” explained Karan Jani, a scientist with the LIGO team at the Georgia Institute of Technology.>
So what’s the big deal?>
The science is novel and splendid – but what really is the significance of these results?>
Foremost: just the sheer thrill at having observed a neutron star merger. There is a reason why we observed four, almost back-to-back gravitational waves from black hole mergers but it took us nearly two years to observe a neutron star merger. “Double neutron star mergers produce gravitational waves of lower amplitude than those produced by double black hole mergers,” Elena Pian, of the Istituto Nazionale di Astrofisica, Rome, and lead author of one of the papers, told The Wire. “Therefore they can be detected only if they are very close by. This dramatically reduces our probability of finding them.”>
Scientists believe something as loud as GW170817 has a probability of occurring only once a decade.The same holds for other phenomena that can emit gravitational waves, like supernovae, making the observation quite rare.>
Second, the burst GRB170817A gives us a vital piece of information about GRBs: this jet was not aimed at us, and we still managed to catch it. The GRB initially looked like every other short burst we see (there are long bursts, too, created by other processes). It was dimmer than the expected calculated brightness of a GRB coming straight for us, and lasted less than two seconds – just like the hundreds of other short GRBs we’ve observed.>
Astronomers have hypothesised that this could be because the jet isn’t directed directly at us. We could have followed up sighting of a dim jet with observations of the afterglow for two days or so, but nothing would have showed up because we weren’t in the path of the jet. Had we been directly in its path, we would have observed the X-rays much quicker.>
It turns out we got lucky. This time, the beam was directed away from us just by 30º or so, so its afterglow reached us, albeit after several days. Given that we’d had a set of gravitational waves with which we could calculate the precise location of the burst, we used the jet’s brightness along with the afterglow data to calculate the angle at which the jet had been shot out.>
“If it was not associated with a gravitational signal, it is likely that GRB170817A would not have been followed up in X-rays, not for 10 days anyway, and the discovery of its delayed X-ray emission would not have been made,” says Pian. This solves what she calls a long-standing fundamental problem in astrophysics: whether dim, short GRBs and brighter, short GRBs are actually the same thing observed from different angles.>
Third, this observation marked the birth of a new chapter for astronomy, titled ‘multi-messenger astronomy’. Imagine you’ve only been able to hear the sound of a tiger: its roar is deep, loud, rumbling. You can tell that the animal is big. The snarly accompaniments would mean it is probably defensive. Then, one day, you see the tiger, and all of a sudden, you are now barraged with information. Those fang-like canines means they rip through meat. The striped coat indicates they blend well with their surroundings, and which means they stalk their prey hidden. Their hind legs are longer than their front legs, which means they jump very well and could probably take down bigger animals. You now know that these creatures are powerful predators. The day you see a tiger hunt will be the day your theories will be tested.>
Today, we’ve seen a tiger hunt.>

A snapshot of a computer simulation showing the evolution of a neutron stars collision. Credit: Stephan Rosswog, Stockholm University, Sweden>
GW170817/GRB170817A was the first joint effort in gravitational interferometry and electromagnetic observations. The electromagnetic information from the kilonova tells us a lot about the new elements that were synthesised. When put in the context of the information from the gravitational waves: they will help us understand stellar evolution better.>
Going back in time>
Astronomers were also able to use GW170817 to calculate the rate of expansion of the universe. Going by how the light from the GRB was distorted and the gravitational waves data giving us the distance of the neutron stars from us, we can tell how fast the neutron stars were away moving from us. Working backwards, we calculate the age of the universe (i.e. ‘how long ago was the universe really, really small’). It’s consistent with our previous estimates: our 13.82-billion-year-old universe is expanding at 67 kilometers per second per megaparsec (a megaparsec is 3.26 million light years) in all directions. The farther an object is, the faster it is moving away from us. “It has worked out brilliantly,” says Pian. “We need to pursue this avenue and make sure all pieces of information that come from the two different channels, gravitational and electromagnetic, are put in context and exploited optimally.”>
And this will happen. We’re already comparably good with gravitational-wave astronomy as we are with electromagnetic astronomy. While the former is still a fledgling field, it is growing quite quickly. The two LIGO and the Virgo interferometers, and the less sensitive GEO600 detector in Germany, will soon be joined by the proposed Kamioka Gravitational Wave Detector in Japan. In 2023, LIGO-India, officially known as Indian Initiative in Gravitational-wave Observations (IndiGO), will join them as well.>
Days after the first gravitational waves detection was publicised, Prime Minister Narendra Modi announced that the Indian project had been approved and a site for the interferometer would be set up in Maharashtra’s Hingoli district. Indian scientists have made valuable contributions to gravitational wave science over the last 30 years. Forty scientists from thirteen Indian institutions are part of the LIGO-Virgo discovery paper, according to IndiGO’s press release. “As a scientist, it is very reassuring to see that India is investing in a field that is just beginning,” said Jani. “Building such mega-science projects allows Indian universities to participate in cutting-edge research, which trickles down to impacting undergraduate education.”>
“It provides a unique opportunity for the Indian scientific community to be a major player in an emerging research frontier,” added P. Ajith, a gravitational wave astrophysicist at the International Centre for Theoretical Sciences, Bengaluru, a member of the LIGO collaboration. “The enormous science and technology challenge involved in this project will motivate some of our best minds to work in India, hopefully putting some break on the brain drain.”>
Also read: India’s LIGO detector has the money it needs, a site in sight, and a completion date too>
A strong gravitational wave detection network, together with electromagnetic observations, would allow us to observe more such wonders of the universe. Pian hopes that “LIGO-Virgo will also detect neutron-star/black-hole mergers that we can follow up with our telescopes and understand the analogies and differences in the nucleosynthesis occurring in them.”>
Will we observe a supernova with the same instruments any time soon? “If a supernova happens in our own galaxy, LIGO and Virgo will be able to detect gravitational waves from it – although supernovae happen in a galaxy once in about 50 years only,” Ajith clarified. “LIGO-Virgo scientists are actively searching for gravitational waves from rapidly rotating neutron stars. We know that they exist since radio telescopes have observed about a thousand of them” in the Milky Way.>
Ultimately, gravitational-wave astronomers want to get back to the Big Bang itself – the same mysterious, explosive event that is thought to have birthed our universe. For about the next 380,000 years after the bang, all the electromagnetic energy was ‘locked in’ with matter particles, not freely moving around. Information about what happened in this time was thus not recorded in the electromagnetic energy, so even though it is loose around us today and detectable in various forms, they don’t tell us any stories about the early universe. However, the gravitational energy in the first 380,000 years could tell us something. Gravitational waves can’t be stopped by anything in their path. They travel the spacetime, even though they get weaker and weaker, as long as they have the spacetime continuum itself.>
‘Catching’ these supremely feeble gravitational waves would require detectors many kilometres long, if not longer – but the day we do catch them… That day, to rephrase William Blake, we’ll see a world in a grain of cosmic sand, and hold infinity in the palm of our hand.>